A Matrix Toolbox for MALDI Imaging Mass Spectrometry
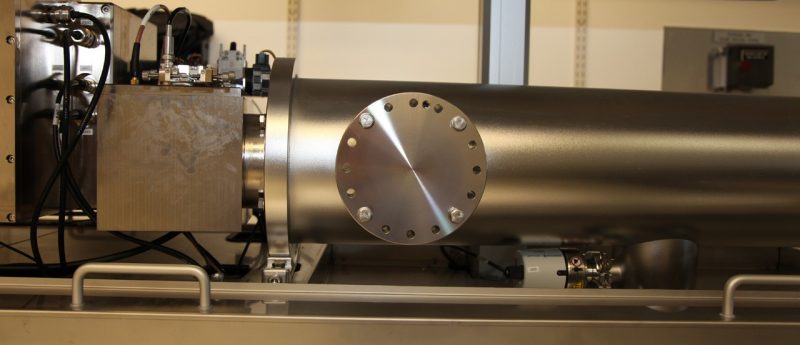
The early 20th century ushered the discovery of the electron, elemental isotopes, and the use of mass spectrometry as a tool to study the elements. As this technology advanced, the study of small, volatile molecules became feasible. However, it would be more than half a century before techniques evolved to analyze large, non-volatile biomolecules, such as proteins. A significant difficulty persisted: the development of a mechanism to volatilize and ionize these molecules in an intact state, without reducing them to fragments [1,2]. Two methods ultimately surfaced to accomplish the analysis of large molecules (>10 kDa) by mass spectrometry – electrospray ionization, pioneered by John Fenn [3], and laser desorption techniques. The latter consists of matrix-assisted laser desorption/ionization (MALDI) developed by Franz Hillenkamp and Michael Karas [4,5] and soft laser desorption developed by Koichi Tanaka [6,7]. These achievements and their proceeding influence on the technology of mass spectrometry would ultimately transform our knowledge about proteins, physiology, and disease [8]. For their work, Fenn and Tanaka each were awarded a quarter of the 2002 Nobel Prize in Chemistry, with the other half going to Kurt Wüthrich for NMR analysis of large biomolecules [9]. This article will focus on MALDI, a form of laser desorption/ionization in which a matrix (e.g., a small organic acid) can be mixed at a high ratio to sample and dried to a crystalline state in order to assist desorption and ionization of the sample analytes [10]. Upon irradiation, the matrix absorbs the energy of the laser and transfers it to the sample, protecting the analytes from the laser energy and facilitating their desorption and ionization as intact molecules [2]. A principle from these early experiments still remains today; the right combination of instrumentation and sample preparation for the analyte of interest are critical for a successful analysis [1,2].
Matrix selection: A key sample preparation step
Early studies of large biomolecules used nicotinic acid [5] or a combination of metal nanoparticles and glycerin [6,7] to obtain spectra of proteins from 10-100 kDa. Today, a commonly used set of MALDI matrices has evolved for successful use with specific analyte classes. For most studies, the analyte is mixed with the matrix prior to drying on a MALDI target. Imaging mass spectrometry (IMS) has largely adapted these matrices; however, there are relevant imaging-specific considerations and applications required to preserve the spatial relationship of the molecules being analyzed, which are discussed below. Table 1 lists examples of effective matrices and provides imaging examples, but it is by no means exhaustive [11]. For additional analytes, such as oligonucleotides and sugars, please see the literature reviews cited in Table 1.
Matrix selection varies among analyte classes. For example, α-cyano-4-hydroxycinnamic acid is a standard choice for peptides. Two matrices are often used for protein analysis, 3,5-dimethoxy-4-hydroxycinnamic acid and 2,5-dihydroxyacetophenone. Empirical findings show that the first is more reliable for spotting applications while the latter is preferred for sublimation and spray-coating. Additionally, 2,5-dihydroxyacetophenone provides increased sensitivity for proteins [12]. A range of matrices exists for small molecule analysis due to the diversity of analytes within this class. For matrix selection here, it is important to examine the mass differences between analytes of interest and the matrix peaks, which are often seen in the low mass range of a MALDI-MS spectrum. The analysis of certain lipid classes can be informative in both positive and negative ion mode. As such, there are matrices for both applications: 1,5-diaminonapthalene is successful in negative ion mode and 2,5-dihydroxyacetophenone is useful in positive ion mode. The matrix 2,5-dihydroxybenzoic acid achieves good results in both modes.
Table 1: MALDI IMS matrices
Analyte class | Effective Matrices | References* |
Peptides | α-cyano-4-hydroxycinnamic acid (CHCA) | [13] |
Proteins | 3,5-dimethoxy-4-hydroxycinnamic acid (sinapinic acid, SA) 2,5-dihydroxyacetophenone (DHA) |
[13] [12,14] |
Lipids | 1,5-diaminonapthalene (DAN) 2,5-dihydroxyacetophenone (DHA) 2,5-dihydroxybenzoic acid (DHB) |
[14,15] [14] [16,17] |
Small molecules | 2,4,6-trihydroxyacetophenone (THAP) α-cyano-4-hydroxycinnamic acid (CHCA) 2,5-dihydroxybenzoic acid (DHB) |
[18,19] [19] [19,20] |
*Listed are examples of imaging applications. For reviews, please see references [10,21,22].
Matrix application
For imaging experiments, several matrix application methods exist. Sublimation is a dry application method (without solvent) of matrix onto a tissue section or MALDI target. This technique was first demonstrated for lipid analysis [23] and has since been applied to protein analysis by incorporating a rehydration (recrystallization) step [24]. Sublimation can be advantageous over wet matrix applications (involving solvent) since it minimizes delocalization of the analytes [23]. Spray-coating and spotting represent two methods for wet matrix application. Several different apparatuses exist for spraying matrix over tissues, and this can be performed manually or using automation [10]. Independent of the device used, an important aim during spraying coating is to achieve a uniform layer of matrix without analyte delocalization. Both sublimation and spraying are suitable to high-spatial resolution imaging, with resolution limited by the matrix crystal and laser spot sizes. When spotting matrix onto tissues, spatial resolution is limited by the size of the matrix droplet placed on the tissue. This approach is often performed in an automated fashion for histology-directed imaging, and it is a valuable technique that allows precise control of matrix onto a tissue area of interest (e.g., a cell-type or disease specific region) [10].
Recently, pre-coating of matrix onto MALDI target slides has also been demonstrated for both lipids [25] and proteins [26]. The slide can be coated with matrix, either via sublimation or spray-coating, and a tissue section is placed onto the pre-coated slide. Excellent signal is obtained in these experiments, similar to traditional post-coating techniques. Pre-coated slides have the potential to facilitate high-throughput imaging in a clinical setting; they offer reproducibility and efficient sample preparation [26].
Additional considerations
Once a matrix has been selected, additional considerations remain. For applications where matrix is applied as a solution (e.g., spray coating and spotting), extraction of the analytes of interest can be further optimized by solvent choice. For example, imaging of hydrophobic membrane proteins is often achieved using a high organic matrix solution [27,28], which differs from the typical 50–70% organic solution [10]. Additives to a matrix solution can also enhance analysis. For example, the derivatization agent 4-hydroxy-3-methoxycinnamaldehyde was pre-coated onto a slide prior to matrix application to facilitate image acquisition of the anti-tuberculosis drug isoniazid [29]. These techniques assist in targeting analytes of interest in a complex sample. Matrix crystal size and matrix stability are also important to imaging experiments. In order to achieve quality images, the matrix crystal must be smaller than the desired spatial resolution and uniformly distributed across the tissue section. Acquisition of high spatial resolution images across large tissue sections can sometimes take several hours. In these situations, it is important to select a matrix that will remain stable under vacuum for the duration of the acquisition.
The preceding discussion provides an overview of typical practices and considerations encountered with human and animal tissue sections in the context of biomedical applications. However, the various possible combinations of matrices, solvents, and sample preparation techniques provide a broad working range in which image acquisition can be optimized for an extensive array of analytes. This attribute positions MALDI IMS as a powerful utility across diverse fields of study [1].
Upcoming Feature: Key Considerations for Clinical IMS Instrumentation
References
1. Markides K, Gräslund A. Mass spectrometry (MS) and nuclear magnetic resonance (NMR) applied to biological macromoluecules. 2002 Nobel Prize in Chemistry – Advanced Information. Nobelprize.org Nobel Media AB 2014. (2002). Available from: http://www.nobelprize.org/nobel_prizes/chemistry/laureates/2002/advanced-chemistryprize2002.pdf
2. Hillenkamp F, Karas M. Chapter 12. Mass spectrometry of peptides and proteins by matrix-assisted ultraviolet laser desorption/ionization. Methods in Enzynmology. Academic Press, 193, 280–295 (1990).
3. Fenn JB, Mann M, Meng CK, Wong SF, Whitehouse CM. Electrospray ionization for mass spectrometry of large biomolecules. Science. 246(4926), 64–71 (1989).
4. Karas M, Bachmann D, Hillenkamp F. Influence of the wavelength in high-irradiance ultraviolet laser desorption mass spectrometry of organic molecules. Anal. Chem. 57(14), 2935–2939 (1985).
5. Karas M, Hillenkamp F. Laser desorption ionization of proteins with molecular masses exceeding 10,000 daltons. Anal. Chem. 60(20), 2299–2301 (1988).
6. Tanaka K, Waki H, Ido Y, et al. Protein and polymer analyses up to m/z 100 000 by laser ionization time-of-flight mass spectrometry. Rapid Commun. Mass Spectrom. 2(8), 151–153 (1988).
7. Yoshida T, Tanaka K, Ido Y, Akita S, Yoshida Y. Detection of high mass molecular ions by laser desorption time-of-flight mass spectrometry. J. Mass Spectrom. Soc. Jpn. 36(2), 59–69 (1988).
8. Nobelprize.org. Nobel Media AB 2014. The 2002 Nobel Prize in Chemistry – Popular Information. Available from: http://www.nobelprize.org/nobel_prizes/chemistry/laureates/2002/popular.html.
9. Williamson MP, Havel TF, Wüthrich K. Solution conformation of proteinase inhibitor IIA from bull seminal plasma by 1H nuclear magnetic resonance and distance geometry. J. Mol. Biol. 182(2), 295–315 (1985).
10. Norris JL, Caprioli RM. Analysis of tissue specimens by matrix-assisted laser desorption/ionization imaging mass spectrometry in biological and clinical research. Chem. Rev. 113(4), 2309–2342 (2013).
11. Reyzer ML. Matrix Application. Advanced Imaging Mass Spectrometry. Short Course and Workshop. Vanderbilt University, Nashville, TN, 113–131 (2015).
12. Zavalin A, Yang J, Hayden K, Vestal M, Caprioli RM. Tissue protein imaging at 1 μm laser spot diameter for high spatial resolution and high imaging speed using transmission geometry MALDI TOF MS. Anal. Bioanal. Chem. 407(8), 2337–2342 (2015).
13. Schwartz SA, Reyzer ML, Caprioli RM. Direct tissue analysis using matrix-assisted laser desorption/ionization mass spectrometry: practical aspects of sample preparation. J. Mass Spectrom. 38(7), 699–708 (2003).
14. Anderson DMG, Spraggins JM, Rose KL, Schey KL. High spatial resolution imaging mass spectrometry of human optic nerve lipids and proteins. J. Am. Soc. Mass Spectrom. 26(6), 940–947 (2015).
15. de Macedo CS, Anderson DM, Pascarelli BM, et al. MALDI imaging reveals lipid changes in the skin of leprosy patients before and after multidrug therapy (MDT). J. Mass Spectrom. 50(12), 1374–1385 (2015).
16. Anderson DMG, Mills D, Spraggins J, Lambert WS, Calkins DJ, Schey KL. High-resolution matrix-assisted laser desorption ionization-imaging mass spectrometry of lipids in rodent optic nerve tissue. Mol. Vis. 19, 581–592 (2013).
17. Angel PM, Spraggins JM, Baldwin HS, Caprioli R. Enhanced sensitivity for high spatial resolution lipid analysis by negative ion mode matrix assisted laser desorption ionization imaging mass spectrometry. Anal. Chem. 84(3), 1557–1564 (2012).
18. Chumbley CW, Reyzer ML, Allen JL, et al. Absolute quantitative MALDI imaging mass spectrometry: A case of rifampicin in liver tissues. Anal. Chem. 88(4), 2392–2398 (2016).
19. Prideaux B, Via LE, Zimmerman MD, et al. The association between sterilizing activity and drug distribution into tuberculosis lesions. Nat. Med. 21(10), 1223–1227 (2015).
20. Castellino S, Groseclose MR, Sigafoos J, et al. Central nervous system disposition and metabolism of fosdevirine (GSK2248761), a non-nucleoside reverse transcriptase inhibitor: An LC-MS and matrix-assisted laser desorption/ionization imaging MS investigation into central nervous system toxicity. Chem. Res. Toxicol. 26(2), 241–251 (2013).
21. Kaletaş BK, van der Wiel IM, Stauber J, et al. Sample preparation issues for tissue imaging by imaging MS. Proteomics. 9(10), 2622–2633 (2009).
22. Chughtai K, Heeren RMA. Mass spectrometric imaging for biomedical tissue analysis. Chem. Rev. 110(5), 3237–3277 (2010).
23. Hankin JA, Barkley RM, Murphy RC. Sublimation as a method of matrix application for mass spectrometric imaging. J. Am. Soc. Mass Spectrom. 18(9), 1646–1652 (2007).
24. Yang J, Caprioli RM. Matrix sublimation/recrystallization for imaging proteins by mass spectrometry at high spatial resolution. Anal. Chem. 83(14), 5728–5734 (2011).
25. Yang J, Caprioli RM. Matrix pre-coated targets for direct lipid analysis and imaging of tissue. Anal. Chem. 85(5), 2907–2912 (2013).
26. Yang J, Caprioli RM. Matrix pre-coated targets for high throughput MALDI imaging of proteins. J. Mass Spectrom. 49(5), 417–422 (2014).
27. Grey AC, Chaurand P, Caprioli RM, Schey KL. MALDI imaging mass spectrometry of integral membrane proteins from ocular lens and retinal tissue. J. Proteome Res. 8(7), 3278–3283 (2009).
28. Nicklay JJ, Harris GA, Schey KL, Caprioli RM. MALDI imaging and in situ identification of integral membrane proteins from rat brain tissue sections. Anal. Chem. 85(15), 7191–7196 (2013).
29. Manier ML, Reyzer ML, Goh A, et al. Reagent precoated targets for rapid in-tissue derivatization of the anti-tuberculosis drug isoniazid followed by MALDI imaging mass spectrometry. J. Am. Soc. Mass Spectrom. 22(8), 1409–1419 (2011).